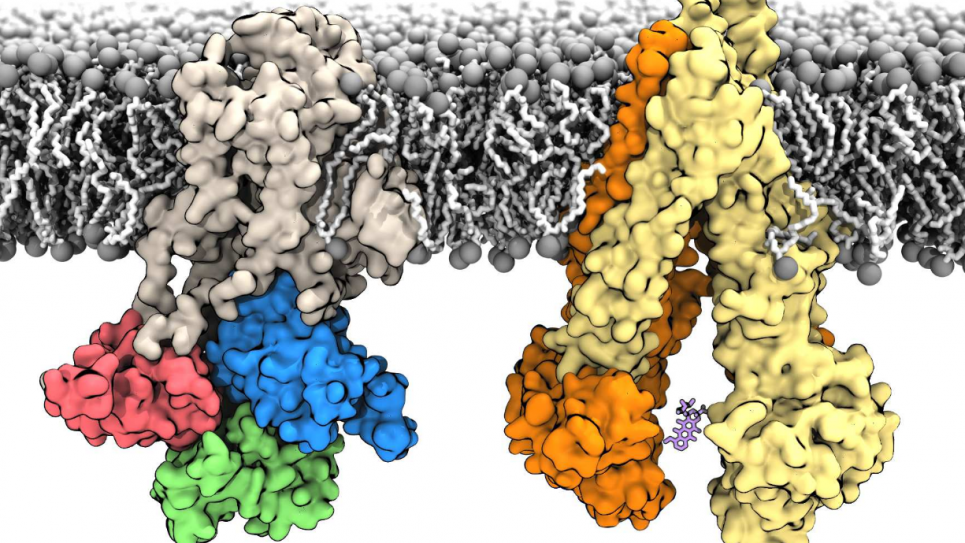
Membrane transport proteins are a unique class of biological macromolecular machines that play critical roles in numerous cellular processes. A distinct feature of these molecular machines is the involvement of large protein conformational changes, often in response to, or in concert with, the altered surrounding chemical environment. A complete understanding of how these proteins carry out their function will thus rely heavily on characterizing these conformational transitions. This work will utilize computational models to provide both detailed visualizations of large protein motions as well as quantitative predictions into the energetics of these processes.
Membrane transport proteins are a unique class of biological macromolecular machines that play critical roles in numerous cellular processes. A distinct feature of these molecular machines is the involvement of large protein conformational changes, often in response to, or in concert with, the altered surrounding chemical environment. A complete understanding of how these proteins carry out their function will thus rely heavily on characterizing these conformational transitions. However, such conformational transition pathways are inherently challenging to determine directly from experiments due to the involvement of transient, short-lived states. Progress on this front will ultimately be possible only by maintaining a strong link between computational and experimental efforts.
This work will utilize computational models to provide both detailed visualizations of large protein motions as well as quantitative predictions into the energetics of these processes.
The main focus will be the determination of the free energy landscapes underlying the function of two large membrane transporters, the Ca2+ATPase (SERCA) and the P-glycoprotein multidrug resistance transporter (PGP). Considerable progress has aleady been made in discovering the transition pathways of these systems using string method simulations; this is a substantial achievement in its own right and has led to several insights. However, a more complete understanding of their mechanism requires a quantitative characterization of these pathways and the free energy landscapes that govern motions along them. This vision can be achieved by leveraging the information from classical molecular dynamics (MD) simulations of atomic-resolution models. Our preliminary results put us in a very strong position to move forward with this exciting project.
NAMD is a widely used MD simulation engine developed by key members of the proposed team. It employs a hybrid force spatial decomposition exploiting the prioritized message-driven execution capabilities of the Charm++ parallel runtime system developed by co-PI Kale. This approach achieves fast, scalable performance by overlapping short-range calculations with long-range PME communication and is amenable to both traditional fixed charge models as well as state-of-the-art polarizable force fields Schulten and Kale shared the 2012 Sidney Fernbach Award for their collaboration on NAMD development. The main computational strategy to be used here for free energy landscape determination is to employ a wide range of multiple-copy algorithms (MCAs), such as umbrella sampling Hamiltonian replica-exchange methodologies; a robust and general implementation of MCAs was recently made available in NAMD. In brief, multiple concurrent NAMD instances are launched with internal partitions of Charm++ and located continuously within a single communication world.